Introduction: Why Understanding the Properties of High-Temperature Alloys Is Essential
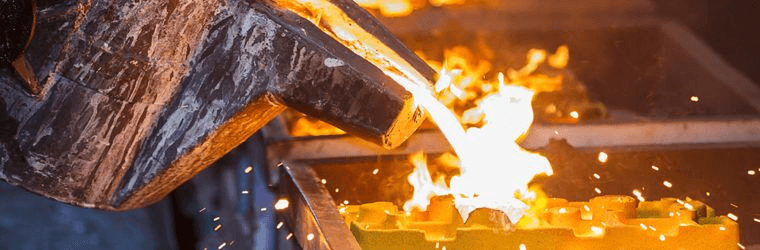
In the world of engineering and advanced manufacturing, high-temperature alloys hold an unmatched position of importance. Why? Because when the heat is on—quite literally—these materials must perform under the most punishing conditions. From powering jet engines to withstanding the searing temperatures inside industrial furnaces, high-temperature alloys are the unsung heroes that keep modern industries moving. But here’s the thing: not all high-temperature alloys are created equal, and selecting the right one for your application is a lot trickier than it seems.
Let’s get straight to it—what makes high-temperature alloys so remarkable? It’s all in their properties. These materials are designed to withstand not only extreme heat but also the stresses, oxidation, and corrosion that come along with it. But here’s the catch: their performance is tied directly to their composition, microstructure, and the conditions they’re exposed to. If you’re an engineer, technician, or decision-maker, understanding these properties isn’t just a “nice-to-have”; it’s absolutely essential for ensuring safety, efficiency, and cost-effectiveness in your operations.
Still with me? Great! Here’s where it gets interesting: high-temperature alloys aren’t a one-size-fits-all solution. Their thermal stability, creep resistance, and oxidation resistance vary widely depending on the alloying elements and processing techniques used. That’s why we’re diving deep into the science and real-world applications of these materials in this guide. By the end, you’ll have the tools to make informed decisions, avoid costly mistakes, and unlock the true potential of high-temperature alloys in your projects. Ready to dig in? Let’s get started!
Thermal Stability: The Backbone of High-Temperature Alloys
Thermal stability is the cornerstone of a high-temperature alloy’s performance. But what does thermal stability actually mean? In simple terms, it’s the ability of a material to maintain its mechanical properties and resist structural changes when exposed to elevated temperatures. This might sound straightforward, but achieving thermal stability requires a delicate balance of alloy composition, heat treatment, and microstructural design.
Take nickel-based superalloys, for instance. These materials owe their incredible thermal stability to a fine dispersion of precipitates such as gamma-prime (Ni3Al) phases within their matrix. Why is this important? Because these precipitates act as barriers to dislocation movement, which means the alloy can maintain its strength even as the temperature rises. However, there’s a catch: these precipitates are only effective up to a certain temperature range. Beyond that, they start to coarsen, weakening the alloy.
Now, let’s talk numbers. Thermal stability isn’t just a theoretical concept; it’s rigorously tested in the lab. Engineers rely on parameters like the service temperature range and creep-rupture strength to assess how well an alloy will perform in real-world conditions. For example, an alloy designed for jet engine turbine blades must withstand temperatures exceeding 1,000°C while maintaining its shape and strength. This isn’t just impressive—it’s downright essential for the safe and efficient operation of the engine.
But here’s where it gets even more fascinating: the role of alloying elements. Chromium, aluminum, and titanium are commonly added to high-temperature alloys to enhance their thermal stability. How do they work their magic? By stabilizing the microstructure and forming protective oxide layers that prevent further degradation. However, the trade-off is that these elements can sometimes reduce the alloy’s ductility or machinability, creating a balancing act for metallurgists.
Before we move on, let’s sum it all up. Thermal stability is what makes high-temperature alloys capable of operating in extreme environments. It’s achieved through a combination of advanced metallurgy, rigorous testing, and precise engineering. And while it might seem like a niche topic, understanding thermal stability is key to unlocking the full potential of these incredible materials. Ready to dive deeper? Let’s explore the next property: oxidation resistance.
Oxidation Resistance: Defending Against Heat-Induced Corrosion
Oxidation resistance is another critical property of high-temperature alloys. What makes it so important? When metals are exposed to high temperatures in environments rich in oxygen, they tend to form oxides on their surface. While this might sound harmless, unchecked oxidation can lead to material degradation, reduced mechanical strength, and ultimately, failure of the component. High-temperature alloys are specifically engineered to resist this process, ensuring longevity and reliability.
The secret behind oxidation resistance lies in the alloy’s chemistry. Here’s how it works: certain alloying elements, such as chromium, aluminum, and silicon, react with oxygen to form a stable, protective oxide layer on the surface. This layer acts as a barrier, preventing further oxidation from penetrating deeper into the material. Chromium, for instance, forms a chromium oxide (Cr2O3) film, which is both adherent and self-healing, making it a popular choice for high-temperature environments.
But let’s not oversimplify. Oxidation resistance is not a one-size-fits-all feature. Different applications require different levels of protection. For example, alloys used in gas turbines face intense thermal and chemical stresses, demanding an oxide layer that can withstand high-velocity gases and temperature fluctuations. In contrast, heat exchangers in chemical plants might prioritize resistance to corrosive gases over mechanical strength.
Now, here’s a fascinating twist: oxidation resistance isn’t just about the alloy’s composition. The manufacturing process plays a huge role too. Techniques like surface coatings and thermal barrier applications can significantly enhance an alloy’s resistance to oxidation. For instance, aluminum-based coatings are often applied to turbine components to provide an additional layer of protection.
Let’s wrap this up: oxidation resistance is the first line of defense for high-temperature alloys against the relentless assault of heat and oxygen. It’s a property that ensures these materials perform reliably in extreme environments, whether in the belly of a jet engine or the core of a power plant. Up next, we’ll tackle a property that’s equally important: mechanical strength at high temperatures.
Mechanical Strength at High Temperatures: Withstanding the Pressure
Why is mechanical strength at high temperatures a game-changer? When components are exposed to elevated temperatures, they must maintain their load-bearing capacity without deforming or failing. High-temperature alloys are specifically engineered to excel in these conditions, making them indispensable in industries like aerospace, automotive, and power generation.
Let’s start with the basics. Mechanical strength at high temperatures is evaluated using two key metrics: tensile strength and creep resistance. Tensile strength measures an alloy’s ability to withstand pulling forces, while creep resistance assesses its ability to resist long-term deformation under constant stress. Together, these properties determine an alloy’s suitability for high-temperature applications.
Take jet engine turbine blades, for instance. These components operate at temperatures exceeding 1,200°C and must endure both mechanical loads and rotational stresses. How do high-temperature alloys handle this? By leveraging their unique microstructures. Nickel-based superalloys, for example, use gamma-prime precipitates to block dislocation movement, ensuring the alloy retains its strength even under extreme conditions.
But there’s more to the story. The role of grain structure cannot be overlooked. Fine-grained alloys tend to offer better creep resistance, while coarse-grained or single-crystal alloys excel in applications requiring high tensile strength. This balance between grain size and mechanical performance is a key consideration for metallurgists.
And here’s something you might not know: mechanical strength isn’t just about the material itself. Environmental factors play a big role too. For example, the presence of corrosive gases or fluctuating temperatures can accelerate material degradation, reducing its effective strength. That’s why engineers often pair high-temperature alloys with protective coatings or cooling systems to extend their service life.
To sum it up, mechanical strength at high temperatures is the backbone of any high-temperature alloy’s performance. It ensures these materials can handle the toughest conditions without losing their integrity. Let’s move forward to another critical property: fatigue resistance.
Fatigue Resistance: Surviving Cyclic Stresses
When it comes to high-temperature applications, fatigue resistance is often the silent hero. Why is this property so critical? In many industrial scenarios, components are subjected to repeated cycles of stress and temperature fluctuations. Over time, these cyclic loads can cause cracks to form and propagate, eventually leading to failure. Fatigue resistance ensures that high-temperature alloys can withstand these stresses over prolonged periods.
The science behind fatigue resistance is both fascinating and complex. At its core, it’s all about microstructural integrity. Alloys with fine-grained structures or reinforcing precipitates are better equipped to resist fatigue. These features act as barriers, preventing the initiation and growth of microcracks under cyclic loading.
But there’s more to consider. Surface conditions also play a huge role in fatigue resistance. Polished surfaces, for instance, are less prone to crack initiation compared to rough or irregular surfaces. That’s why many high-temperature components undergo surface treatments like shot peening or polishing to enhance their fatigue life.
And let’s not forget about temperature. High temperatures exacerbate fatigue by accelerating microstructural changes and oxidation. This is particularly true for components like turbine disks and combustion chambers, where thermal cycling is the norm. Engineers address this challenge by selecting alloys with superior high-temperature fatigue properties and using advanced cooling techniques.
Before we move on, here’s the big takeaway: fatigue resistance is what keeps high-temperature alloys working reliably under cyclic stresses. It’s a property that’s often overlooked but is absolutely essential for long-term performance. Let’s now explore how weldability and machinability impact the usability of these alloys.
Weldability and Machinability: The Practical Challenges
High-temperature alloys are marvels of engineering, but they come with their own set of challenges. What’s the main issue here? Their superior properties often make them difficult to weld or machine, creating hurdles during fabrication and assembly. However, understanding these challenges—and how to overcome them—is crucial for leveraging the full potential of these materials.
Weldability, for instance, can be a tricky affair. Why? High-temperature alloys are prone to issues like cracking, distortion, and loss of mechanical properties during welding. Nickel-based superalloys, for example, have a narrow temperature range for welding, making them particularly challenging. To address this, engineers often use specialized welding techniques like TIG (tungsten inert gas) welding or electron beam welding, which provide better control over heat input and minimize defects.
Machinability is another story. High-temperature alloys are notoriously tough and resistant to cutting. While these properties are desirable in service, they make machining a time-consuming and expensive process. Tools made from carbide or polycrystalline diamond are often used to handle the extreme wear and heat generated during machining.
And here’s a fascinating twist: some alloys are designed specifically for improved machinability without compromising their high-temperature properties. How is this achieved? By tweaking the alloy’s composition or adding elements like sulfur or lead to improve chip formation.
In conclusion, weldability and machinability are practical challenges that must be addressed when working with high-temperature alloys. By understanding these issues and using advanced techniques, engineers can unlock the full potential of these incredible materials. Up next, we’ll take a look at the future of high-temperature alloys.
Future Trends in High-Temperature Alloys
The world of high-temperature alloys is constantly evolving. What’s driving these changes? Advances in technology, growing environmental concerns, and the increasing demands of modern industries are all pushing the boundaries of what these materials can achieve.
One exciting trend is the development of new materials like intermetallic compounds and ceramic-matrix composites. Why are these materials gaining attention? Because they offer superior high-temperature performance compared to traditional alloys. For instance, ceramic-matrix composites are lighter and more heat-resistant, making them ideal for aerospace applications.
Additive manufacturing is another game-changer. How does it impact high-temperature alloys? By enabling the creation of complex geometries and reducing material waste. This technology is particularly useful for producing turbine blades and other components that require precise customization.
Finally, there’s a growing focus on sustainability. How are researchers addressing this? By developing alloys with lower environmental impact and improving recycling processes. These efforts are crucial for reducing the carbon footprint of industries that rely heavily on high-temperature alloys.
To sum up, the future of high-temperature alloys is bright, with innovations paving the way for more efficient and sustainable solutions. Let’s conclude this guide by summarizing the key takeaways.
Conclusion
High-temperature alloys are the backbone of modern engineering, offering unmatched performance in extreme environments. From thermal stability and oxidation resistance to mechanical strength and fatigue resistance, these materials are engineered to tackle the toughest challenges. While they come with fabrication hurdles like welding and machining difficulties, advancements in technology and materials science are making them more versatile than ever. As industries evolve, so too will these alloys, ensuring they remain a cornerstone of innovation and reliability. Thanks for exploring this guide—here’s to making the best-informed decisions for your next high-temperature application!
FAQs About High-Temperature Alloys
Q: What are the main uses of high-temperature alloys?
High-temperature alloys are used in aerospace, automotive, and energy sectors for components like turbine blades, exhaust systems, and heat exchangers.
Q: Which alloy is best for extreme temperatures?
Nickel-based superalloys are often the top choice due to their excellent thermal stability and oxidation resistance.
Q: How do I choose the right high-temperature alloy for my application?
Consider factors like operating temperature, mechanical stresses, and environmental conditions. Consult with a materials expert for tailored recommendations.